How big is a synapse?
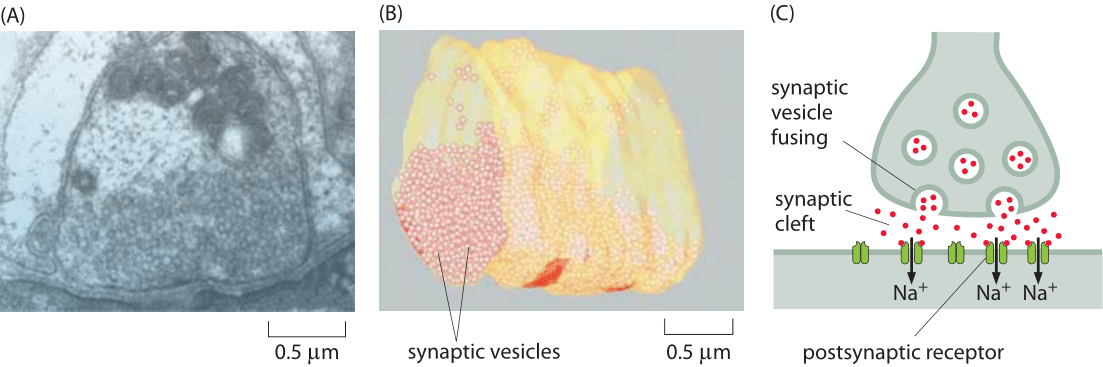
Figure 1: Structure of a neuromuscular junction. (A) Electron microscopy image of a nerve terminal and its synapse with a neighboring cell in a neuromuscular junction. (B) Cryo-electron microscopy reconstruction image of a fraction of the presynaptic neuron showing the synaptic vesicles it harbors for future release. (C) Schematic of a synapse. Note that the synaptic cleft, vesicles etc. are not drawn to scale. (A, B adapted from S. O. Rizzoli and W. J. Betz, Nat. Rev. Neurosci., 6:57, 2005.)
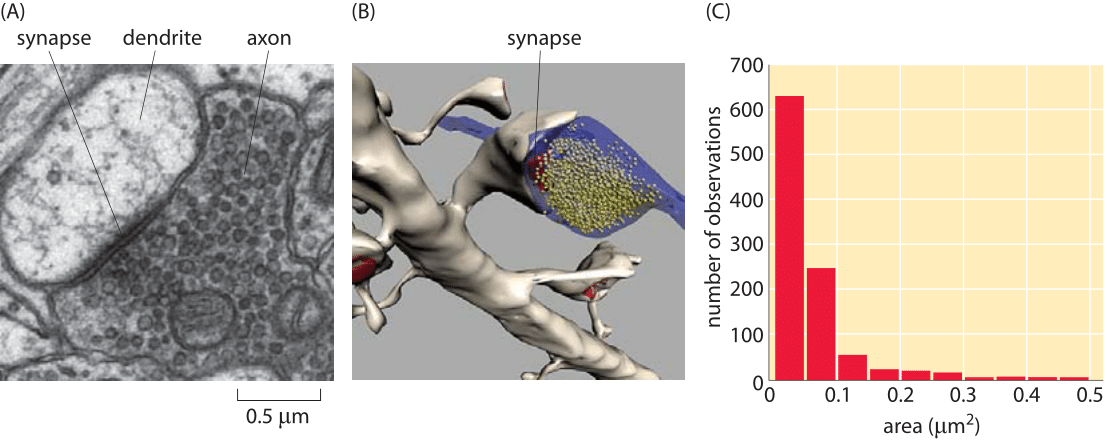
Figure 2: Size of synapses in the brain. (A) Electron microscopy image of a synapse between an axon and a dendrite. (B) Reconstruction of a synapse like that shown in (A) illustrating the synaptic vesicles. (C) Distribution of synapse sizes as measured using electron microscopy. (Figures courtesy of Linnaea Ostroff)
So far in the book, we have mainly focused on the sizes of individual cells and the molecules, macromolecular complexes and organelles within them. Multicellularity, however, is all about partnerships between cells. A beautiful example of our own multicellularity is provided by the cells in our nervous system. These cells are part of a vast and complex array of interactions that are only now beginning to be mapped. The seat of interactions between neighboring neurons are synapses, the interface between cells in which small protrusions adopt a kissing configuration as seen in Figures 1 and 2 for the cases of a neuromuscular junction and a synapse in the brain, respectively. These synapses are responsible for the propagation of information from one neuron to the next. Interestingly, information transmission in the nervous system is partly electrical and partly chemical. That is, when an action potential travels along a nerve, it does so by transiently changing the transmembrane potential from its highly negative resting value to a nearly equal positive potential. When the action potential reaches the synapse, this leads to vesicle fusion and subsequent release of chemical signals (neurotransmitters) which induce channel gating in the neighboring cell with which it has formed the synapse. This results in turn in an action potential in the neighboring cell. As seen in Figures 1 and 2, the synapse is composed of a pre-synaptic terminal on the axon of the transmitting neuron and a post-synaptic terminal with a so-called synaptic cleft between them. The total number of such synapses in the human brain has been vaguely stated to be in the range of 1013-1015 (BNID 106138, 100693), with every cubic millimeter of cerebral cortex having about a billion such synapses (BNID 109245).
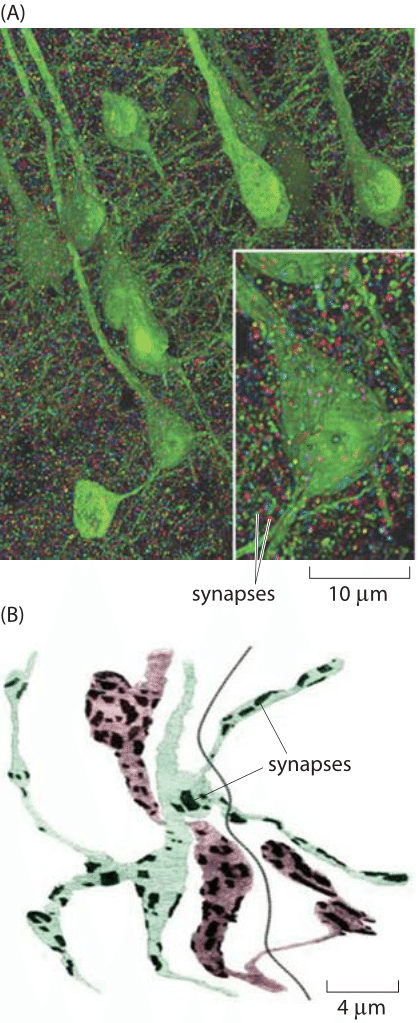
Figure 3: Images of the synapse. (A) Volume rendering of the somatosensory cortex of a mouse. The synaptic marker synapsin has been immunolabeled making it possible to see the individual synapsin puncta connecting neurons labeled in green. The individual synapses were rendered in random colors. (B) Reconstruction of two axons from Drosophila showing the location of synaptic connections (dark patches). Color is used to distinguish the two cells. The dark line is the boundary between the two muscles that are in contact with the axons. (A adapted from D. Kleinfeld et al., J. Neurosci.,31:16215, 2011; B adapted from S. O. Rizzoli and W. J. Betz, Nat. Rev. Neuroscience, 6:57, 2005.)
Recent experimental developments have now made it possible to revisit order of magnitude estimates like those given above based on volume renderings of synapses such as those shown in Figure 3. Based on such experiments, we have begun to garner a multiscale structural view of the connections between different neurons. Further, these maps are providing an increasingly specific view of the chemical diversity found in synapses. That is, depending upon which specific cell type is under consideration, the complement of proteins present in the synapse region will be different. At the scale of individual synapses, a close up view of the roughly 1 μm box into which most synapses fit is now in hand. Both classic electron microscopy and its three-dimensional tomographic extensions paint a beautiful picture of synapses with their complement of synaptic vesicles. Using tomographic techniques and a combination of light and electron microscopy, scientists have mapped out the rich network of connections between neurons, including their complements of synaptic vesicles. Figures 1 and 2 show several different views of the distributions of these micron-sized synapses on individual neurons. Indeed, Figure 2C gives a precise quantitative picture of the range of synaptic sizes in the brain. To get a sense of scale, the reader is invited to recall the size of a bacterium with its ≈1 μm3 volume, meaning that each of these synapses is roughly the size of a bacterium (BNID 111086).
To better understand the intimate connection between structure and function in the cells of the nervous system, consider the processes that take place when we read a book. First, photons reflected from the page are absorbed by rhodopsin in the photoreceptors of our eyes. This photon absorption results in a signal cascade in the photoreceptor of the retina, that culminates in the release of neurotransmitters at the synapse. Specifically, the synaptic vesicles fuse with the membrane of the pre-synaptic cell as shown in Figures 1C and 2A (though the microscopy image in Figure 1 shows a neuromuscular junction and not the synapse of a photoreceptor) and release 103-104 neurotransmitter molecules from each vesicle (BNID 108622, 108623, 102777). Common neurotransmitters are glutamate, used in about 90% of synapses (BNID 108663), as well as acetylcholine and GABA which are packed at a high concentration of 100-200 mM in the synaptic vesicles (BNID 102777). Each vesicle is about 10-5 μm3 in volume (BNID 102776), so our rule of thumb that 1 nM concentration in 1 μm3 is about 1 molecule enables us to verify that there are indeed about 1000 neurotransmitter molecules per vesicle. These molecules then diffuse into the synaptic cleft and bind receptors on the post-synaptic cell surface. The signal propagating to our brain is carried by electric action potentials within neurons and relayed from one neuron to the next by similar synaptic fusion events. Vesicle release is triggered by 102-104 Ca2+ ions (BNID 103549). The energy expended per vesicle release has been estimated to be about 105 ATP/vesicle (BNID 108667). Synapses are cleared within about 1 ms preparing the way for future communication. Rapid clearing is essential as neuronal firings can reach rates of over 100 times per second (BNID 107124), though the average firing rate is estimated to be 1-10 Hz in the cortex (BNID 108670). The delay created by the time it take a neurotransmitter to diffuse across the synaptic cleft (not drawn to scale in the schematic of Figure 1) is part of the response time of humans to any reflex or neural action of any sort. Conveniently, it takes less than 1 ms to traverse the 20-40 nm synaptic divide (BNID 100721, 108451) as the reader can easily verify after reading the vignette on “What are the time scales for diffusion in cells?”. Interestingly, this can be compared to the time it takes for the action potential to propagate down a nerve which is on the ms time scales as discussed in the vignette on “How fast are electrical signals propagated in cells?”.